Class 10 Science and Technology Part 1 notes are meticulously written in my own words, drawing from extensive research and thorough reading of the entire Maharashtra State board textbook of Science and Technology Part 1. Crafted to aid both in-depth understanding and efficient last-minute revision, these notes cover every chapter with clarity and focus. Whether you’re studying new concepts, revisiting key points, or preparing for exams, these notes will serve as a reliable resource, simplifying complex ideas and highlighting essential topics. Here, you will find all the chapter notes in one place, tailored to meet the needs of Class 10 students for the Science and Technology Part 1 syllabus.
Gravitation
Gravitation chapter explores the concept of gravitation, a force that acts between any two objects with mass. Discovered by Sir Isaac Newton, gravitation is responsible for various natural phenomena, such as the falling of objects, planetary orbits, and even the behavior of tides. We’ll cover key topics like gravitational force, circular motion, Kepler’s laws, and concepts like free fall and escape velocity. Understanding these concepts will deepen your grasp of the forces governing both earthly and cosmic interactions.
What is Gravitation?
Gravitation is the force of attraction that acts between any two objects with mass. Newton’s discovery of this force helped explain why objects fall toward the Earth and why planets orbit the Sun. This force is universal, meaning it applies to all objects, regardless of size or distance.
Common Question: Why don’t we notice gravitational attraction between everyday objects?
This is because the gravitational force is significantly stronger between massive objects like the Earth and smaller objects like an apple. For small objects close to each other, the force is too weak to notice.
Newton’s Universal Law of Gravitation
Newton’s Universal Law of Gravitation states that every object in the universe attracts every other object with a force that is directly proportional to the product of their masses and inversely proportional to the square of the distance between them. Mathematically, it’s expressed as:
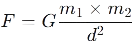
where F is the gravitational force, G is the gravitational constant, and ddd is the distance between the centers of the masses m1 and m2..
Example Question: What happens to the force if the distance between two objects doubles?
The force decreases by a factor of four (since force is inversely proportional to the square of the distance).
Circular Motion and Centripetal Force
Any object moving in a circular path requires a force directed toward the center of the circle, known as centripetal force. This force is essential for maintaining circular motion. For planets orbiting the Sun, gravity provides this centripetal force, keeping them in their orbits.
Student Query: Why don’t planets fly off into space?
The gravitational pull from the Sun acts as the centripetal force, holding the planets in orbit. Without this force, planets would move in a straight line due to inertia.
Kepler’s Laws of Planetary Motion
Johannes Kepler proposed three laws of planetary motion:
- First Law: Planets move in elliptical orbits with the Sun at one focus.
- Second Law: A line segment joining a planet and the Sun sweeps out equal areas during equal intervals of time.
- Third Law: The square of a planet’s orbital period is proportional to the cube of the average distance from the Sun.
Common Question: How did Kepler’s Laws influence Newton?
Newton used Kepler’s observations to formulate his law of gravitation, explaining why planets obey these laws through gravitational attraction.
Acceleration Due to Gravity (g)
The acceleration due to gravity on Earth is approximately 9.8 m/s29.8 \, m/s^29.8m/s2. This acceleration means that objects near Earth’s surface experience a downward pull, causing them to accelerate at this rate when falling freely.
Common Question: Does the value of g change with altitude?
Yes, g decreases as we move further from the Earth’s surface, as the distance from Earth’s center increases.
Free Fall and Gravitational Acceleration
Free fall occurs when an object moves under the influence of gravity alone, with no other forces acting on it. In free fall, objects accelerate downward at 9.8m/s2 (assuming no air resistance).
Common Question: Will a heavy and a light object fall at the same rate?
Yes, in the absence of air resistance, all objects fall at the same rate due to gravity. Galileo demonstrated this concept by dropping objects of different masses from the Leaning Tower of Pisa.
Concept of Weight and Mass
- Mass is the amount of matter in an object, measured in kilograms (kg). Mass is constant and does not change with location.
- Weight is the force with which an object is attracted toward Earth, calculated as W=mg
. Weight varies based on gravitational strength and is a vector directed toward the center of the Earth.
Student Query: Why does your weight differ on the Moon?
The Moon’s gravity is about 1/6th of Earth’s gravity, so an object’s weight on the Moon is much lower than on Earth, even though its mass remains the same.
Escape Velocity
Escape velocity is the minimum speed required for an object to escape Earth’s gravitational pull without further propulsion. On Earth, this velocity is around 11.2km/s.
Common Question: Why does a rocket need to reach escape velocity?
If a rocket reaches escape velocity, it can leave Earth’s gravitational influence and enter space without being pulled back.
Gravitational Potential Energy
Gravitational potential energy is the energy an object possesses due to its position in a gravitational field. Near Earth’s surface, it’s calculated as U=mgh, where h is the height above the ground.
Common Question: Why is potential energy considered negative in space physics?
When considering an object infinitely far from Earth, the potential energy is zero. As it moves closer, the gravitational attraction causes potential energy to become negative, indicating a bound system.
Effect of Gravity on Planets and Satellites
Gravity not only keeps planets in orbit but also governs the motion of moons and artificial satellites. These objects are in a continuous state of free fall toward the planet they orbit, but their high tangential velocity prevents them from falling.
Common Question: Why don’t satellites fall back to Earth?
Satellites are moving at just the right speed to keep falling “around” Earth rather than crashing into it.
Tides and Gravitational Effects of the Moon
The gravitational pull of the Moon causes tides on Earth. High tides occur on the side closest to the Moon and directly opposite it, while low tides occur at points 90 degrees from these areas.
Common Question: How does the Moon’s gravity cause tides?
The Moon’s gravity pulls on Earth’s oceans, creating a bulge of water (high tide) both on the side facing the Moon and the opposite side.
Gravitational Waves
Predicted by Einstein, gravitational waves are ripples in space-time caused by massive objects in motion, such as colliding black holes. These waves carry information about the universe and were first detected in 2015 by the LIGO observatory.
Common Question: Why are gravitational waves important?
Gravitational waves allow scientists to observe cosmic events that don’t emit light, giving us a new way to study the universe.
Conclusion:
The chapter on gravitation unveils the fundamental force that shapes the universe, from everyday objects falling to Earth to the motion of planets and galaxies. Understanding gravitational force, acceleration due to gravity, and concepts like weight, free fall, and escape velocity provides insights into both terrestrial and cosmic phenomena. Mastering these concepts will give students a strong foundation in physics, aiding not only in exams but in understanding the natural world.
Periodic Classification of Elements
Periodic Classification of Elements delves into the systematic organization of elements, known as the periodic classification, which helps in understanding the similarities and differences among elements. Early scientists attempted to group elements based on their properties, leading to classifications like Dobereiner’s Triads, Newlands’ Octaves, Mendeleev’s Periodic Table, and ultimately the Modern Periodic Table. By studying these classifications, students can understand patterns in element properties, periodic trends, and the modern arrangement of elements.
Classification of Elements
Early scientists recognized patterns among elements and classified them to simplify study. Initially, elements were grouped into metals and non-metals based on physical properties. As more elements were discovered, scientists sought a more organized system to reflect properties and behaviors consistently across elements.
Common Question: Why classify elements?
Classification makes it easier to predict properties and reactions of elements based on their groups, aiding in scientific understanding and practical application.
Dobereiner’s Triads
In 1817, German scientist Johann Dobereiner noted that certain elements could be grouped in threes, called triads, with similar chemical properties. He observed that the atomic mass of the middle element in a triad was approximately the average of the other two. An example is the triad of lithium (Li), sodium (Na), and potassium (K).
Common Question: Are all elements arranged in triads?
No, Dobereiner’s Triads only worked for a limited number of elements, so it wasn’t a comprehensive classification system.
Newlands' Law of Octaves
English scientist John Newlands introduced the Law of Octaves in 1866, observing that every eighth element had similar properties when arranged by increasing atomic mass. This arrangement was likened to musical octaves, where notes repeat after every seventh note. Newlands’ approach worked well for lighter elements but failed beyond calcium.
Common Question: Why didn’t Newlands’ law apply to heavier elements?
Newlands’ law did not account for elements that were yet to be discovered or for variations in atomic mass trends among heavier elements, limiting its applicability.
Mendeleev's Periodic Table
Dmitri Mendeleev, a Russian chemist, developed a more comprehensive periodic table between 1869 and 1872. He arranged 63 elements by increasing atomic mass and grouped them by similar properties. Mendeleev’s table had vertical columns called groups and horizontal rows called periods. He also left gaps for undiscovered elements, predicting their properties accurately.
Common Question: Why was Mendeleev’s table revolutionary?
Mendeleev’s predictions about undiscovered elements (like gallium and germanium) were confirmed later, proving the predictive power of his table.
Merits of Mendeleev's Periodic Table
- Prediction of New Elements: Mendeleev left empty spaces in his table, predicting properties of undiscovered elements like “eka-aluminum” (gallium) based on surrounding elements.
- Grouping Based on Properties: Elements with similar chemical behaviors were grouped together, making it easy to study trends.
- Flexibility with Atomic Mass: Mendeleev prioritized grouping similar elements together over strictly following atomic mass order.
Common Question: How did Mendeleev’s table handle inconsistencies?
Mendeleev allowed exceptions to atomic mass order, believing element properties were more important for grouping, which was a key strength of his approach.
Limitations of Mendeleev's Periodic Table
- Isotopes: Isotopes (elements with the same atomic number but different atomic masses) created ambiguity.
- Position of Hydrogen: Hydrogen showed properties similar to both alkali metals and halogens, so its correct placement was unclear.
- Inconsistencies in Atomic Mass Order: Elements like cobalt and nickel didn’t follow the increasing atomic mass rule, creating placement issues.
Common Question: Why was hydrogen’s position challenging?
Hydrogen shares properties with both alkali metals and halogens, so its exact group placement was uncertain.
Modern Periodic Law
With advances in atomic theory, English scientist Henry Moseley revised the periodic classification. He found that atomic number (the number of protons) was a more fundamental property than atomic mass. In 1913, he proposed the Modern Periodic Law, stating: “Properties of elements are a periodic function of their atomic numbers.” This resolved inconsistencies in Mendeleev’s table and laid the foundation for the modern periodic table.
Common Question: Why use atomic number over atomic mass?
Atomic number uniquely identifies elements based on proton count, making it more reliable for organizing elements without exceptions like isotopes.
Structure of the Modern Periodic Table
The modern periodic table is organized by increasing atomic number, with elements grouped into 18 vertical columns (groups) and seven horizontal rows (periods). Elements in the same group have similar properties, and periods indicate the energy levels of electrons.
Key Points:
- Groups 1 and 2: Known as the s-block elements.
- Groups 3 to 12: Transition metals, or d-block elements.
- Groups 13 to 18: p-block elements, including non-metals and noble gases.
- Lanthanides and Actinides: The f-block elements shown separately at the bottom.
Electronic Configuration and the Periodic Table
An element’s position in the periodic table depends on its electron configuration. Elements in the same group have the same number of valence electrons, giving them similar properties. For example, group 1 elements (alkali metals) all have one valence electron.
Common Question: Why are elements in the same group similar?
Elements in a group have the same valence electron configuration, which determines their chemical reactivity.
Periodic Trends in the Modern Periodic Table
Understanding trends within periods and groups helps predict element properties:
- Atomic Size: Decreases across a period due to increased nuclear charge and increases down a group as new electron shells are added.
- Metallic and Non-metallic Character: Metallic character increases down a group (e.g., group 1 metals become more reactive), while non-metallic character increases across a period.
Common Question: Why does atomic size decrease across a period?
Increased nuclear charge pulls electrons closer to the nucleus, reducing atomic radius.
Valency in the Modern Periodic Table
Valency, or the combining capacity of an element, is determined by the number of electrons in its outermost shell. Across a period, valency increases up to group 14 and then decreases. Down a group, valency remains the same as elements share the same number of valence electrons.
Common Qustion: How does valency vary in groups and periods?
Valency is consistent within a group and follows a pattern across periods due to the number of valence electrons.
Metallic and Non-metallic Character in Groups and Periods
- Metals are typically located on the left and center of the periodic table, while non-metals are on the right.
- Moving across a period from left to right, elements become less metallic and more non-metallic.
- Down a group, metallic character increases, meaning elements become more electropositive (tend to lose electrons).
Common Question: Why are non-metals more common on the right side of the table?
Non-metals have higher electronegativity and prefer to gain electrons, making them more stable on the right side of the table where valence shells are nearly filled.
Conclusion:
The periodic classification of elements allows for a clear understanding of patterns in chemical properties and behaviors. From Dobereiner’s Triads to the Modern Periodic Table, each stage in the evolution of the table has contributed to our knowledge of elements. The modern periodic table, organized by atomic number, provides a powerful tool for predicting element properties, reactivity, and behavior in chemical reactions. By understanding periodic trends, students gain insights into both the theoretical and practical aspects of chemistry.
Chemical Reactions and Equations
Chemical Reactions and Equations introduces the fascinating world of chemical reactions, where substances undergo transformations to create new materials. By learning how to write chemical equations, balance them, and understand the types of reactions, students gain a solid foundation in chemistry. This chapter covers definitions, the method for writing and balancing equations, the classification of reactions, and practical examples, enabling students to visualize and understand these concepts deeply.
Definition of a Chemical Reaction
A chemical reaction is a process where one or more substances (reactants) are transformed into different substances (products) by breaking and forming chemical bonds. Chemical reactions result in new properties and compositions in the products compared to the reactants.
Common Question: How can you tell if a chemical reaction has occurred?
Common signs include color change, gas formation, temperature change, and the appearance of a precipitate.
Physical vs. Chemical Changes
- Physical Change: Involves a change in state, shape, or size without altering the chemical composition (e.g., ice melting into water).
- Chemical Change: Involves the formation of new substances with different chemical compositions and properties (e.g., burning wood).
Common Question: Why is dissolving sugar in water a physical change?
Dissolving sugar in water is a physical change because the sugar molecules are dispersed in water, not chemically altered.
Types of Chemical Reactions
Chemical reactions are classified based on the changes in substances, the number of reactants and products, and the type of bond changes. Key types include combination, decomposition, displacement, and double displacement reactions.
Combination Reaction
A combination reaction occurs when two or more reactants combine to form a single product.
- Example: Magnesium combines with oxygen to form magnesium oxide:

Common Question: Why is this reaction called a combination reaction?
It’s called a combination reaction because multiple reactants merge to form one product.
Decomposition Reaction
A decomposition reaction involves a single compound breaking down into two or more simpler substances.
- Example: Calcium carbonate decomposes into calcium oxide and carbon dioxide upon heating:

Common Question: What conditions are needed for a decomposition reaction?
Decomposition reactions often require heat, light, or electricity to initiate the breakdown of compounds.
Displacement Reaction
In a displacement reaction, a more reactive element replaces a less reactive element in a compound.
- Example: Zinc displaces copper from copper sulfate

Common Question: Why does zinc replace copper in this reaction?
Zinc is more reactive than copper, so it displaces copper from copper sulfate.
Double Displacement Reaction
Double displacement reactions involve the exchange of ions between two compounds to form new compounds. One of the products is often a precipitate.
- Example: Silver nitrate reacts with sodium chloride to form silver chloride and sodium nitrate:

Common Question: What makes a reaction double displacement?
In a double displacement reaction, ions switch places between compounds, resulting in new products.
Chemical Equations
A chemical equation is a symbolic representation of a chemical reaction where reactants and products are written with their chemical formulas.
Common Question: What does an arrow in a chemical equation signify?
The arrow points from reactants to products, indicating the direction of the reaction.
Writing Word Equations
A word equation describes the reactants and products of a chemical reaction in words.
- Example: Magnesium + Oxygen → Magnesium Oxide
Writing Chemical Equations
Chemical equations are written using chemical symbols. The reactants are listed on the left side and products on the right side, separated by an arrow.
- Example:

Common Question: Why use symbols instead of words?
Symbols make it easier to understand and balance chemical reactions efficiently.
Balancing Chemical Equations
In a balanced chemical equation, the number of atoms for each element is equal on both sides. Balancing equations follows the Law of Conservation of Mass, which states that mass is conserved in a closed system.
Steps to Balance an Equation:
- Write the equation with the correct formulas.
- Count the atoms of each element on both sides.
- Use coefficients to balance each element one by one.
- Double-check that all elements are balanced.
Common Question: Why do we balance chemical equations?
Balancing ensures that the mass and atoms are conserved according to the Law of Conservation of Mass.
Endothermic and Exothermic Reactions
- Endothermic Reaction: Absorbs heat, making the surroundings cooler (e.g., melting ice).
- Exothermic Reaction: Releases heat, making the surroundings warmer (e.g., burning wood).
Common Question: How can you tell if a reaction is endothermic or exothermic?
If the reaction absorbs heat, it’s endothermic; if it releases heat, it’s exothermic.
Oxidation and Reduction (Redox Reactions)
- Oxidation: The addition of oxygen or the loss of hydrogen.
- Reduction: The removal of oxygen or the addition of hydrogen.
Example: Magnesium oxide forms when magnesium is oxidized:

Common Question: Why are oxidation and reduction reactions often paired?
In redox reactions, one substance is oxidized while another is reduced, allowing electrons to transfer.
Catalysts and their Role in Reactions
A catalyst speeds up a reaction without being consumed. It provides an alternative reaction pathway with lower activation energy.
Common Question: What role does a catalyst play in decomposition?
A catalyst helps decompose reactants faster by lowering the energy needed for the reaction.
Corrosion
Corrosion is the gradual degradation of metals due to oxidation, often seen in iron rusting. Moisture and oxygen are essential for corrosion to occur.
Example: Iron reacts with oxygen and moisture to form rust:

Common Question: Why is corrosion a problem?
Corrosion weakens metals, leading to structural issues and economic loss.
Rancidity
Rancidity occurs when fats and oils oxidize, resulting in an unpleasant smell and taste. Storing food in airtight containers and using antioxidants can prevent rancidity.
Common Question: Why do packaged foods have preservatives?
Preservatives, often antioxidants, prevent rancidity by slowing down oxidation.
Conclusion:
This chapter covers the basics of chemical reactions, from understanding types of reactions to balancing equations. Grasping these concepts will enable students to analyze chemical reactions, understand energy changes, and predict outcomes in both academic and real-life scenarios. Through practice and examples, students can master chemical equations and deepen their understanding of chemistry.
Effects of Electric Current
Effects of Electric Current is about exploring the different effects that electric current has when it flows through various materials. We’ll study energy transfer in electric circuits, the heating and magnetic effects of electric current, and applications of these effects in devices like electric motors and generators. These concepts are foundational for understanding how electric current powers our everyday appliances and how it can be converted into other forms of energy.
Energy Transfer in an Electric Circuit
When an electric current flows through a circuit, energy is transferred from the source (such as a battery or power supply) to components within the circuit. This energy transfer results in different effects, like heating and magnetic fields.
Common Question: What happens to the energy in an electric circuit?
The energy provided by the battery or power source is converted into heat or other forms of energy, depending on the components in the circuit, like resistors or motors.
Heating Effect of Electric Current
When current passes through a conductor with resistance, some electrical energy is converted into heat. This is known as the heating effect of electric current, which is utilized in appliances like electric heaters and light bulbs.
Example:
Consider an electric heater where a high-resistance wire (often made of nichrome) heats up when current flows through it. This heat is then used to warm up the surrounding area.
Common Question: Why do resistors get hot when current flows through them?
Resistors oppose the flow of current, causing energy loss in the form of heat. This heat is proportional to the square of the current, as described by the formula H=I2×R×t (Joule’s Law of Heating).
Joule’s Law of Heating
Joule’s Law states that the heat H produced in a resistor due to current I flowing through it for time t with resistance R is given by:

This law helps explain why heating devices are designed with specific resistances to produce desired amounts of heat.
Common Question: How does current affect the heat produced?
Heat is directly proportional to the square of the current, meaning a small increase in current results in a much larger increase in heat.
Applications of the Heating Effect
- Electric Bulbs: Tungsten filaments heat up and emit light when current flows through them.
- Electric Heaters and Boilers: High-resistance coils convert electrical energy into heat for heating purposes.
- Fuses: Fuse wires melt when excess current flows through them, breaking the circuit to prevent damage.
Common Question: Why is nichrome used in electric heaters?
Nichrome has high resistivity and can withstand high temperatures, making it ideal for producing heat without melting.
Magnetic Effect of Electric Current
When current flows through a conductor, it creates a magnetic field around it. This was discovered by Hans Christian Oersted, who observed that a magnetic needle near a current-carrying wire deflected, proving a relationship between electricity and magnetism.
Example:
Wrapping a current-carrying wire around an iron core creates an electromagnet, which is useful in devices like electric bells and relays.
Common Question: Why does a magnetic field form around a current-carrying wire?
Moving electric charges generate magnetic fields, which is why a current in a wire produces a magnetic field around it.
Right-Hand Thumb Rule
The Right-Hand Thumb Rule helps determine the direction of the magnetic field around a straight current-carrying conductor. If you hold the conductor in your right hand with your thumb pointing in the direction of the current, the curl of your fingers shows the direction of the magnetic field.
Common Question: How is the Right-Hand Thumb Rule useful?
This rule is crucial in predicting the direction of magnetic lines of force, which helps in understanding and designing electromagnets and motors.
Magnetic Field Around a Current-Carrying Circular Loop
When current flows through a circular loop, a magnetic field forms around it. The field lines are circular near the wire and appear straight at the center. The magnetic field strength increases with the number of turns in the coil.
Common Question: What happens to the magnetic field if we add more loops to the coil?
Increasing the number of loops intensifies the magnetic field at the center of the coil.
Electromagnet and Its Applications
An electromagnet is a type of magnet created by wrapping a coil of wire around an iron core and passing current through it. Electromagnets are widely used in electric motors, generators, and other devices requiring controlled magnetic fields.
Common Question: What makes electromagnets useful?
Electromagnets can be turned on or off by controlling the current, making them versatile for applications like cranes for lifting heavy metal objects.
Fleming’s Left-Hand Rule
Fleming’s Left-Hand Rule is used to determine the direction of force on a current-carrying conductor in a magnetic field. If you position your left hand with the thumb, forefinger, and middle finger perpendicular to each other, then:
- Thumb: Direction of force (motion of the conductor)
- Forefinger: Direction of magnetic field
- Middle Finger: Direction of current
Common Question: Why do we need Fleming’s Left-Hand Rule?
This rule helps predict the direction of force, essential in designing electric motors and understanding how they work.
Electric Motor: Principle and Working
An electric motor converts electrical energy into mechanical energy. It works on the principle of electromagnetic induction, where a force is exerted on a current-carrying conductor in a magnetic field, causing it to rotate. The motor consists of a coil placed in a magnetic field and connected to a power source.
Common Question: How does an electric motor work in household appliances?
The motor’s rotation powers fans, mixers, washing machines, etc., by converting electric energy into the mechanical energy needed to move components.
Electromagnetic Induction
Electromagnetic induction is the process of generating an electric current by moving a conductor within a magnetic field or changing the magnetic field around the conductor. Discovered by Michael Faraday, this principle is fundamental for electric generators.
Common Question: How is electromagnetic induction used in generators?
In a generator, a coil moves within a magnetic field, inducing an electric current that can be harnessed as AC or DC power.
Electric Generator
An electric generator converts mechanical energy into electrical energy by rotating a coil within a magnetic field. The rotation causes a change in the magnetic flux through the coil, inducing an alternating current (AC) or direct current (DC), depending on the generator type.
Common Question: What is the main difference between an AC and DC generator?
An AC generator uses slip rings to produce alternating current, while a DC generator uses a split ring to produce direct current.
Conclusion:
The chapter on the effects of electric current highlights how electric current produces heat and magnetism, which can be harnessed in various devices. From electric motors to generators, understanding these principles enables us to use electric current in practical applications effectively. Mastering concepts like electromagnetic induction, Fleming’s rules, and electric circuits equips students to explore advanced topics in electricity and magnetism.
Heat
Heat chapter explores the concept of heat, covering topics like the difference between heat and temperature, methods of heat transfer, specific heat capacity, and phase changes involving latent heat. It also includes real-world phenomena such as the anomalous expansion of water, humidity, and the dew point. Understanding these concepts provides insights into everyday observations, like why metal feels colder than wood and how aquatic life survives in icy waters.
Difference Between Heat and Temperature
- Heat is a form of energy that flows from a hotter object to a cooler one until thermal equilibrium is reached. It is measured in joules (J) or calories (cal).
- Temperature is the measure of the thermal energy of particles in a substance. It determines the direction of heat flow and is measured in degrees Celsius (°C), Kelvin (K), or Fahrenheit (°F).
Common Question: Why does metal feel colder than wood at the same temperature?
Metal conducts heat away from your skin faster than wood due to its high thermal conductivity, making it feel colder.
Methods of Heat Transfer
Heat can be transferred in three primary ways: conduction, convection, and radiation.
- Conduction: Heat transfer within a material or between materials in direct contact. Metals are good conductors, while materials like wood are insulators.
- Convection: Heat transfer in fluids (liquids and gases) where warmer parts of the fluid rise and cooler parts sink, creating a cycle.
- Radiation: Heat transfer through electromagnetic waves, like sunlight reaching Earth.
Common Question: Why does a fan increase the cooling effect?
The fan circulates air, enhancing convection by moving warm air away from your body and bringing in cooler air.
Specific Heat Capacity
pecific heat capacity (c) is the amount of heat required to raise the temperature of 1 kg of a substance by 1°C. It varies between materials.
Formula: Q=m×c×ΔT
Where Q is heat energy, mmm is mass, c is specific heat capacity, and ΔT is the temperature change.
Common Question: Why does water take longer to heat than sand?
Water has a high specific heat capacity, meaning it requires more energy to raise its temperature compared to sand.
Latent Heat and Phase Changes
- Latent Heat is the heat absorbed or released during a phase change at a constant temperature.
- Types of Latent Heat:
- Latent Heat of Fusion: Heat absorbed to convert a solid into a liquid at its melting point.
- Latent Heat of Vaporization: Heat absorbed to convert a liquid into gas at its boiling point.
Example: When ice melts at 0°C, it absorbs latent heat without a temperature increase, transforming into water.
Common Question: Why doesn’t the temperature change during melting or boiling?
During phase changes, the heat energy is used to overcome molecular bonds rather than raising temperature.
Temperature vs. Time Graph for Phase Changes
The graph demonstrates how temperature remains constant during phase transitions, such as from ice to water at 0°C and water to steam at 100°C.
Common Question: What happens to the energy during constant temperature?
The energy goes into changing the state by breaking or forming molecular bonds, rather than increasing temperature.
Anomalous Expansion of Water
Water behaves unusually between 0°C and 4°C, contracting instead of expanding as it heats up from 0°C to 4°C. This property is called the anomalous expansion of water and leads to maximum density at 4°C.
Common Question: Why does ice float on water?
Water is most dense at 4°C, so ice, which is less dense, floats. This property helps insulate aquatic life during winter.
Regelation
Regelation is the phenomenon where ice melts under pressure and refreezes once the pressure is removed. This occurs because the melting point of ice decreases with increased pressure.
Example: Pressing two ice cubes together can make them stick as they undergo regelation.
Common Question: How does regelation help in ice skating?
The pressure from the skater’s blade momentarily melts the ice, creating a thin water layer that reduces friction.
Humidity and Dew Point
- Humidity refers to the amount of water vapor in the air. High humidity levels make the air feel warmer.
- Dew Point is the temperature at which air becomes saturated with water vapor, leading to condensation (e.g., dew on grass).
Common Question: Why do we feel hotter on humid days?
High humidity slows down the evaporation of sweat, making it harder for the body to cool down.
Heat Units: Joules and Calories
- Joule (J): SI unit of heat energy.
- Calorie (cal): Amount of heat needed to raise the temperature of 1 g of water by 1°C.
Conversion: 1 cal = 4.18 J.
Common Question: Why use both Joules and Calories?
Calories are commonly used in food and biological contexts, while Joules are preferred in scientific contexts.
Principle of Heat Exchange
n an isolated system, heat lost by a hotter object equals heat gained by a cooler object until they reach the same temperature.
Formula: Heat lost=Heat gained
Common Question: Why does a cold spoon warm up in hot soup?
The spoon absorbs heat from the soup until both reach thermal equilibrium.
Calorimeter and Specific Heat Measurement
A calorimeter is used to measure the specific heat capacity of substances. When a hot object is placed in water inside the calorimeter, heat exchange occurs until equilibrium is reached, allowing calculation of specific heat.
Common Question: How does a calorimeter prevent heat loss?
The calorimeter’s insulating material minimizes heat exchange with the surroundings, ensuring accurate measurements.
Application of Specific Heat in Daily Life
Materials with high specific heat, like water, are used for cooling systems due to their ability to absorb large amounts of heat. Metals with low specific heat are preferred in cooking utensils because they heat up quickly.
Example Question: Why is water effective for cooling engines?
Water’s high specific heat allows it to absorb and transfer large amounts of heat, preventing engine overheating.
Hope’s Apparatus and the Anomalous Expansion of Water
Hope’s Apparatus is used to study the anomalous behavior of water. When a mixture of ice and salt is placed in the apparatus, the water’s density and temperature change in a unique pattern, showcasing maximum density at 4°C.
Common Question: Why is this property crucial for aquatic life?
In cold climates, the water at the lake’s bottom remains at 4°C, allowing aquatic life to survive in liquid water despite freezing temperatures above.
Formation of Dew and Frost
Dew forms when moist air cools below its dew point, causing water vapor to condense on surfaces. Frost forms similarly, but at temperatures below freezing.
Example: Dew on grass in the morning is common when the ground cools overnight.
Common Question: Why do we see more dew on cool mornings?
On cool mornings, the air temperature drops below the dew point, resulting in condensation on surfaces.
Conclusion:
The chapter on heat offers a comprehensive understanding of how thermal energy affects matter through concepts like specific heat, latent heat, and temperature changes. By exploring topics such as heat transfer methods, anomalous expansion of water, and humidity, students gain practical insights into phenomena they observe in daily life. Mastering these concepts is essential for understanding not only physical science but also various applications in technology and environmental science.
Refraction of Light
Refraction of Light chapter covers the concept of refraction, where light changes its direction when passing from one medium to another, like air to water. We’ll study the laws governing refraction, refractive index, and real-world applications such as mirages, rainbows, and the twinkling of stars. Understanding refraction deepens our grasp of optical phenomena and how lenses and prisms work.
What is Refraction of Light?
Refraction is the bending of light as it passes from one transparent medium into another, due to a change in its speed. When light moves from a medium with a lower refractive index (like air) to a higher one (like glass), it bends toward the normal, and vice versa.
Common Question: Why does a pencil look bent in water?
This is due to refraction; light changes direction as it moves from water to air, making the submerged part of the pencil appear displaced.
Laws of Refraction
- First Law: The incident ray, refracted ray, and normal all lie in the same plane.
- Second Law (Snell’s Law): The ratio of the sine of the angle of incidence (iii) to the sine of the angle of refraction (rrr) is constant for two given media:
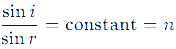
where n is the refractive index of the second medium with respect to the first.
Common Question: What does Snell’s Law tell us?
Snell’s Law quantifies how much light bends when moving between two materials with different refractive indices.
Refractive Index
The refractive index is a measure of how much a medium can bend light. It is defined as the ratio of the speed of light in a vacuum to the speed of light in the medium:

Common Question: Why does light slow down in glass?
The dense molecular structure of glass obstructs light, reducing its speed and increasing refraction.
Absolute and Relative Refractive Index
- Absolute Refractive Index: Refers to a material’s refractive index with respect to vacuum.
- Relative Refractive Index: The ratio of the speed of light between two different media.
Example: The refractive index of water is about 1.33, meaning light travels 1.33 times slower in water than in a vacuum.
Total Internal Reflection
Total internal reflection occurs when light moves from a denser to a rarer medium and the angle of incidence exceeds a certain limit, known as the critical angle. Beyond this angle, light reflects entirely within the denser medium rather than refracting.
Example: Optical fibers use total internal reflection to transmit light over long distances without losing intensity.
Common Question: What is the practical use of total internal reflection?
It’s used in devices like periscopes, binoculars, and fiber-optic cables to efficiently channel light.
Critical Angle and Conditions for Total Internal Reflection
The critical angle is the minimum angle of incidence at which total internal reflection occurs. It depends on the refractive indices of the two media:
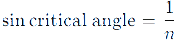
Common Question: Why do diamonds sparkle?
Diamonds have a high refractive index, resulting in a small critical angle, which causes multiple total internal reflections, enhancing their sparkle.
Atmospheric Refraction and Twinkling of Stars
Atmospheric refraction occurs when light from stars passes through layers of Earth’s atmosphere with varying densities. This causes the star’s light path to bend continuously, making it appear to twinkle.
Common Question: Why don’t planets twinkle?
Planets appear as small discs rather than points of light, so atmospheric refraction affects their whole image, reducing the twinkling effect.
Mirage: An Optical Illusion
A mirage is caused by the refraction and total internal reflection of light in layers of air with varying temperatures. Hot surfaces, like a desert, heat the air above them, creating a gradient that bends light upward, making it look like there’s water on the ground.
Common Question: Why does a mirage look like water?
The upward bending of light creates the illusion of a reflection, similar to what we see on a water surface.
Dispersion of Light
Dispersion occurs when white light separates into its component colors upon passing through a prism. Each color has a different wavelength, with red bending the least and violet bending the most.
Example: This separation creates a spectrum of colors, visible in phenomena like rainbows.
Common Question: Why do we see different colors after light passes through a prism?
Different colors travel at slightly different speeds in the prism, causing them to spread out and form a spectrum.
Formation of a Rainbow
A rainbow is formed through dispersion, refraction, and total internal reflection of sunlight in water droplets. Sunlight enters the droplet, splits into colors, reflects internally, and exits, creating a circular arc of colors.
Common Question: Why are rainbows curved?
The curved path of light rays inside spherical water droplets gives the rainbow its arc shape.
Apparent Shift in the Position of Objects in Water
Objects under water appear closer to the surface than they actually are due to refraction. Light from the object bends away from the normal as it moves from water to air, causing an apparent shift.
Example: A fish viewed from above water appears closer to the surface than its real position.
Common Question: Why does this happen?
Refraction at the water-air boundary shifts the light path, creating an illusion of a shallower depth.
Refraction in Lenses
Lenses, such as convex and concave lenses, bend light rays to form images. Convex lenses converge light rays, creating real or virtual images, while concave lenses diverge light rays, creating only virtual images.
Common Question: How do eyeglasses help us see clearly?
Lenses in eyeglasses adjust the focus of light onto the retina, correcting vision by compensating for refractive errors in the eye.
Optical Density vs. Physical Density
Optical density refers to a material’s ability to slow down light, whereas physical density measures mass per unit volume. Materials like glass are optically dense (slow down light) but may not be physically dense.
Common Question: Can a material be optically dense but not heavy?
Yes, materials like diamond are optically dense but not always heavy; they bend light significantly due to high refractive index.
Refraction and Apparent Depth in Water
The phenomenon of apparent depth explains why objects in water appear shallower than they are. Refraction at the water’s surface makes the path of light bend, affecting perception.
Example: If you place a coin at the bottom of a clear water bowl, it appears to rise closer to the observer.
Common Question: Why does an object in water look closer?
Light bends at the water-air interface, making the image seem elevated compared to its actual position.
Conclusion:
The chapter on refraction of light provides insights into the behaviors of light as it travels through various media, influencing real-world optical effects and technologies. By exploring concepts like refraction, total internal reflection, dispersion, and critical angle, students gain a foundational understanding of phenomena seen in everyday life—from rainbows to mirages and eyeglasses. Mastering these principles opens up pathways to exploring more advanced optics and applications in science and technology.
Lenses
Lenses chapter explores how transparent, curved surfaces refract light to form images. Lenses are essential in everyday optical devices like spectacles, cameras, microscopes, and telescopes. In this chapter, we study the types of lenses, their properties, the rules of image formation, and applications in correcting vision. By mastering these concepts, students will understand how lenses manipulate light to achieve practical functions.
What is a Lens?
A lens is a transparent medium bound by two curved surfaces. It refracts light to form images by converging or diverging light rays.
- Convex Lens (Converging Lens): Thicker at the center, it converges light rays to a point.
- Concave Lens (Diverging Lens): Thinner at the center, it diverges light rays, making them appear to originate from a single point.
Key Terms in Lenses
- Centre of Curvature (C): The centers of the spherical surfaces forming the lens. A double lens has two centers of curvature, C1 and C2.
- Radius of Curvature (R): The radius of the spheres whose surfaces form the lens.
- Principal Axis: An imaginary straight line passing through the centers of curvature and the optical center.
- Optical Center (O): A point inside the lens where light rays pass through without deviation.
- Principal Focus (F):
- Convex Lens: Parallel rays converge at a focus on the other side of the lens.
- Concave Lens: Parallel rays diverge, appearing to originate from a focus on the same side as the light source.
- Focal Length (f): Distance between the optical center and the principal focus.
Rules for Image Formation by Lenses
- A ray parallel to the principal axis passes through the principal focus after refraction.
- A ray passing through the principal focus becomes parallel to the principal axis after refraction.
- A ray passing through the optical center does not deviate.
Image Formation by Convex Lenses
Using ray diagrams, we can predict the position, size, and nature of the image formed:
- Object at Infinity: Image forms at F2 point-sized, real, and inverted.
- Object Beyond 2F1: Image forms between F2 and 2F2, smaller, real, and inverted.
- Object at 2F1: Image forms at 2F2, same size, real, and inverted.
- Object Between F1 and 2F1: Image forms beyond 2F2, larger, real, and inverted.
- Object at F1: Image forms at infinity, very large, real, and inverted.
- Object Between F1 and O: Image forms on the same side as the object, larger, virtual, and erect.
Common Question: Why does a convex lens produce a real and inverted image for most object positions?
The rays actually converge after refraction, forming a real image. Since the rays intersect, the image appears inverted.
Image Formation by Concave Lenses
- Object at Infinity: Image forms at F1, point-sized, virtual, and erect.
- Object Anywhere Else: Image forms between O and F1, smaller, virtual, and erect.
Example Question: Why does a concave lens always form a virtual image?
The rays diverge after refraction, and the image is formed by extending the refracted rays backward.
Lens Formula and Magnification
Lens Formula:

where f is the focal length, v is the image distance, and u is the object distance.
Magnification (M):
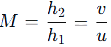
where h2 is the height of the image, and h1 is the height of the object.
Power of a Lens
The power (P) of a lens is its ability to converge or diverge light.
- Formula:
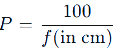
Unit: Diopter (D).
- A convex lens has positive power, while a concave lens has negative power.
Common Question: A lens has a focal length of 50 cm. What is its power?
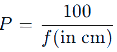
Applications of Lenses
- Convex Lenses:
- Simple microscopes for magnifying objects.
- Cameras to focus light for photographs.
- Projectors to enlarge images onto a screen.
- Correcting farsightedness in spectacles.
- Concave Lenses:
- Peep holes in doors for a wider field of view.
- Correcting nearsightedness in spectacles.
Vision Defects and Correction
- Nearsightedness (Myopia):
- Distant objects appear blurry.
- Corrected using concave lenses.
- Farsightedness (Hypermetropia):
- Nearby objects appear blurry.
- Corrected using convex lenses.
- Presbyopia:
- Loss of lens elasticity due to aging, causing difficulty in seeing nearby objects.
- Corrected using bifocal lenses.
Common Question: Why do we use bifocal lenses for presbyopia?
Bifocal lenses combine convex and concave lenses to address both farsightedness and nearsightedness.
Human Eye and Lenses
The human eye uses a convex lens to focus light on the retina.
- Power of Accommodation: The eye lens adjusts its focal length to focus on objects at various distances.
- Defects:
- Corrected using lenses tailored to the individual’s specific requirements.
Common Question: How does the eye focus on near and far objects?
The ciliary muscles adjust the lens’s shape, changing its focal length to focus light on the retina.
Optical Instruments Using Lenses
- Simple Microscope: A convex lens magnifies small objects.
- Compound Microscope: Combines two lenses for higher magnification.
- Telescope: Uses convex lenses or mirrors to observe distant objects.
- Cameras and Projectors: Focus light to form images on film or screens.
Conclusion:
The chapter on lenses highlights their ability to refract light and form images. From optical instruments to correcting vision, lenses play an integral role in both science and daily life. By mastering concepts like image formation, power of lenses, and their applications, students can appreciate the wide-ranging impact of lenses in understanding and manipulating light.
Metallurgy
Metallurgy chapter explores the processes involved in extracting metals from their natural ores and refining them for practical use. Metallurgy is a vital field that helps us obtain metals used in construction, transportation, electronics, and more. This chapter covers topics like the physical and chemical properties of metals and nonmetals, methods of concentration, extraction and refining of metals, corrosion, and its prevention. By understanding these principles, students will appreciate the science behind materials that shape our world.
What is Metallurgy?
Metallurgy is the science of extracting metals from ores, refining them, and preparing them for practical use. It encompasses various physical and chemical processes to obtain pure metals from naturally occurring compounds.
Common Question: Why do we need metallurgy?
Metals rarely occur in their pure form in nature. Metallurgy enables us to extract and refine these metals to meet industrial and daily needs.
Physical Properties of Metals and Nonmetals
Metals:
- Exist mainly in solid state (exceptions: mercury and gallium are liquid at room temperature).
- Shiny and lustrous.
- Good conductors of heat and electricity.
- Malleable (can be hammered into sheets) and ductile (can be drawn into wires).
- Have high melting and boiling points (e.g., tungsten: 3422°C).
- Produce a ringing sound when struck (sonorous).
Nonmetals:
- May exist in solid, liquid, or gaseous states (exception: bromine is a liquid).
- Dull in appearance (iodine crystals are shiny).
- Poor conductors of heat and electricity (graphite is an exception).
- Brittle and non-malleable.
- Low melting and boiling points.
Common Question: Why is mercury liquid at room temperature?
Mercury’s atomic structure and weak interatomic forces prevent it from solidifying at room temperature.
Chemical Properties of Metals and Nonmetals
Reactions of Metals:
- With Oxygen: Form metal oxides. Example: 2Mg+O2→2MgO
- With Water: Reactive metals like sodium release hydrogen gas. Example: 2Na+2H2O→2NaOH+H2
- With Acids: Produce hydrogen gas and form salts. Example: Zn+2HCl→ZnCl2+H2
2. Reactions of Nonmetals:
- With Oxygen: Form acidic or neutral oxides. Example: S+O2→SO2
- Generally do not react with water or acids (exceptions: halogens react with water).
Common Question: Why are nonmetals poor conductors of electricity?
Nonmetals lack free electrons, which are required for electrical conduction.
Reactivity Series of Metals
The reactivity series arranges metals in order of their reactivity:
- Highly Reactive: Potassium, Sodium.
- Moderately Reactive: Iron, Zinc.
- Less Reactive: Gold, Silver.
Common Question: Why is gold found in its pure form in nature?
Gold is unreactive and does not combine with other elements, allowing it to remain pure.
Extraction of Metals
- Concentration of Ores:
- Gravitational Method: Heavy ore particles are separated from gangue using gravity.
- Magnetic Separation: Magnetic ores are separated using electromagnetic rollers.
- Froth Floatation: Ores like zinc sulfide are separated by froth formed with oil and air.
2. Reduction of Ores:
- Electrolytic Reduction: Highly reactive metals like sodium are extracted by electrolysis.
- Roasting and Calcination:
- Roasting: Heating sulfide ores in air to form oxides.
- Calcination: Heating carbonate ores in limited air.
Common Question: What is the difference between roasting and calcination?
Roasting uses oxygen, while calcination occurs in limited air without oxygen.
3. Refining of Metals:
- Electrolytic Refining: Impure metal is refined using electrolysis. Example: Copper refining uses copper electrodes and an acidic solution.
Corrosion and Prevention
What is Corrosion? Corrosion is the gradual destruction of metals due to environmental reactions, like rusting of iron or patination of copper.
Prevention Methods:
- Galvanization: Coating iron with zinc.
- Electroplating: Coating one metal with another (e.g., gold-plated jewelry).
- Alloying: Mixing metals to reduce corrosion (e.g., stainless steel).
Common Question: Why doesn’t stainless steel rust?
Stainless steel contains chromium, which forms a protective oxide layer, preventing rust.
Key Processes in Metallurgy
Electrolysis of Alumina:
- Used for extracting aluminium from bauxite.
- Alumina is dissolved in cryolite to reduce melting point.
- Aluminium is deposited at the cathode.
2. Thermit Reaction:
- Aluminium reduces iron oxide to produce molten iron.
- Used in welding processes.
Common Question: Why is cryolite used in aluminium extraction?
Cryolite lowers the melting point of alumina, reducing energy consumption during electrolysis.
Summary Table
Process | Definition | Example |
---|
Gravitational Method | Separating ores based on density | Gold extraction |
Magnetic Separation | Separating magnetic from nonmagnetic ores | Cassiterite (tin ore) |
Froth Floatation | Using oil and air to extract sulfide ores | Zinc blende (ZnSZnSZnS) |
Electrolytic Reduction | Using electricity to extract metals from molten ores | Sodium, Aluminium |
Roasting | Heating sulfide ores in air | Zinc sulfide to zinc oxide |
Calcination | Heating carbonate ores in limited air | Calcium carbonate to calcium oxide |
Conclusion:
Metallurgy is the backbone of material science, enabling the extraction and refining of metals that drive technology and development. Understanding the principles of metallurgy not only prepares students for exams but also fosters an appreciation for the processes that shape our industrial world.
Carbon Compounds
Carbon Compounds chapter explores the incredible versatility of carbon, the basis of organic chemistry. From its unique bonding properties to its role in forming complex molecules, carbon forms the backbone of numerous compounds essential for life and technology. The chapter dives into types of compounds, bonding in carbon compounds, hydrocarbons, functional groups, homologous series, and key reactions such as combustion, oxidation, and esterification. Understanding these concepts provides insight into both everyday materials and advanced chemical processes.Lorem ipsum dolor sit amet, consectetur adipiscing elit. Ut elit tellus, luctus nec ullamcorper mattis, pulvinar dapibus leo.
Types of Compounds
- Organic Compounds: Contain carbon; examples include glucose, ethanol, and methane.
- Inorganic Compounds: Do not typically include carbon except in cases like CO₂, CO, carbonates, and carbides.
Common Question: Why is carbon dioxide considered inorganic despite containing carbon?
Carbon dioxide does not have C-H bonds, a hallmark of organic compounds.
Unique Properties of Carbon
Catenation:
- The ability of carbon atoms to bond with each other, forming chains, rings, and networks.
- Results in diverse structures like straight chains, branched chains, and cyclic compounds.
Tetravalency:
- Carbon forms four covalent bonds with other atoms, enabling it to combine with elements like H, O, N, and halogens.
Multiple Bonding:
- Carbon can form single, double, and triple bonds, adding to the diversity of compounds.
Isomerism:
- Compounds with the same molecular formula but different structures. Example: Butane and isobutane (C₄H₁₀).
Common Question: What allows carbon to form millions of compounds?
Carbon’s catenation, tetravalency, and ability to form multiple bonds.
Bonding in Carbon Compounds
Covalent Bonds:
- Carbon achieves stability by sharing electrons.
- Represented by electron-dot structures or line structures.
Types of Bonds:
- Single Bond: In ethane (C₂H₆), carbon atoms are connected by single bonds.
- Double Bond: In ethene (C₂H₄), two carbon atoms share two pairs of electrons.
- Triple Bond: In ethyne (C₂H₂), carbon atoms share three pairs of electrons.
Hydrocarbons
- Definition: Compounds containing only carbon and hydrogen.
- Types:
- Saturated Hydrocarbons (Alkanes): Contain single bonds. Example: Methane (CH₄).
- Unsaturated Hydrocarbons: Contain double or triple bonds.
- Alkenes: Ethene (C₂H₄).
- Alkynes: Ethyne (C₂H₂).
Functional Groups and Homologous Series
Functional Groups:
- Groups of atoms that determine chemical properties.
- Examples:
- Hydroxyl group (-OH) in alcohols.
- Carboxyl group (-COOH) in acids.
Homologous Series:
- A group of compounds with the same functional group and similar properties.
- Example: Alcohols (CH₃OH, C₂H₅OH, etc.).
- Characteristics:
- Same general formula.
- Gradation in physical properties.
Common Query: Why do members of a homologous series have similar properties?
They share the same functional group, which governs their chemical behavior.
Important Carbon Compounds
Ethanol (C₂H₅OH):
- Properties: Colorless liquid, soluble in water, boiling point 78°C.
- Uses: Solvent, antiseptic, fuel additive.
- Reactions:
- With sodium: Forms hydrogen gas and sodium ethoxide.
- Dehydration: Forms ethene when heated with concentrated sulfuric acid.
Ethanoic Acid (CH₃COOH):
- Properties: Sour liquid, boiling point 118°C.
- Uses: Preservative in vinegar, industrial solvent.
- Reactions:
- Neutralization: Reacts with NaOH to form sodium acetate.
- Esterification: Forms esters with alcohols, e.g., ethyl ethanoate.
Reactions of Carbon Compounds
Combustion:
- Carbon compounds burn in oxygen to produce CO₂, H₂O, heat, and light.
Example: CH₄ + 2O₂ → CO₂ + 2H₂O.
- Carbon compounds burn in oxygen to produce CO₂, H₂O, heat, and light.
Oxidation:
- Example: Ethanol oxidizes to ethanoic acid using potassium permanganate.
Addition Reactions:
- Unsaturated compounds (alkenes, alkynes) react with halogens or hydrogen.
Example: Ethene + H₂ → Ethane.
- Unsaturated compounds (alkenes, alkynes) react with halogens or hydrogen.
Substitution Reactions:
- Saturated hydrocarbons react with halogens in the presence of sunlight.
Example: CH₄ + Cl₂ → CH₃Cl + HCl.
- Saturated hydrocarbons react with halogens in the presence of sunlight.
Common Question: How does a saturated hydrocarbon react with chlorine?
Through substitution, where hydrogen atoms are replaced by chlorine atoms.
Macromolecules and Polymers
- Definition: Large molecules formed by repeating small units (monomers).
- Natural Polymers:
- Examples: Starch, cellulose, proteins, DNA.
- Synthetic Polymers:
- Examples: Polyethylene, PVC, Teflon.
- Uses: Plastics, non-stick cookware, fibers.
Nomenclature of Carbon Compounds
IUPAC Rules:
- Identify the longest chain of carbon atoms.
- Number the chain, giving priority to functional groups.
- Add suffixes (e.g., -ol for alcohols, -oic acid for carboxylic acids).
Examples:
- CH₃OH: Methanol.
- CH₃COOH: Ethanoic acid.
- CH₃CH₂CH₂OH: Propanol.
Conclusion:
The chapter on carbon compounds highlights the versatility and importance of carbon in forming diverse compounds essential to life and industry. From hydrocarbons to polymers, and from functional groups to chemical reactions, this chapter lays the foundation for understanding organic chemistry’s vast scope. Mastering these concepts enables students to appreciate both the molecular world and its real-world applications.
Space Missions
Space Missions chapter explores mankind’s journey into space, from the first artificial satellite to advanced interplanetary explorations. It delves into the importance of satellites for communication, navigation, and weather forecasting, as well as missions to the Moon, Mars, and beyond. The chapter also highlights the role of organizations like ISRO and NASA, the challenges of space exploration, and the need to manage space debris. Understanding these concepts provides insights into humanity’s quest to unravel the mysteries of the universe.
What is Space?
- Definition: Space begins where Earth’s atmosphere ends, a vast, near-vacuum region filled with stars, planets, and galaxies.
- Difference Between Space and Sky: The sky is the atmosphere visible from Earth, while space lies beyond it.
Common Question: Why is space exploration important?
Space exploration helps us understand the origins of the universe, develop new technologies, and improve life on Earth through satellite applications.
Need and Importance of Space Missions
- Communication: Satellites enable instant global communication via the internet, television, and telephony.
- Weather Monitoring: Satellites help predict weather, track storms, and monitor climate changes.
- Navigation: Systems like GPS use satellites to provide accurate location data.
- Disaster Management: Satellites aid in predicting and responding to natural disasters like earthquakes and floods.
- Scientific Exploration: Missions expand our knowledge of celestial bodies and the universe’s origins.
Artificial Satellites
- Definition: Manmade objects placed in orbit around Earth or other planets.
- Examples: INSAT, GSAT, Sputnik.
- Key Components: Solar panels for power, instruments for signal transmission, and cameras for observation.
Common Question: How do artificial satellites function?
Satellites orbit Earth, using solar panels for energy and onboard instruments to collect and transmit data to ground stations.
Types of Satellites
- Weather Satellites: Study and predict weather. Example: INSAT series.
- Communication Satellites: Enable global communication. Example: GSAT series.
- Navigational Satellites: Provide precise location data. Example: IRNSS.
- Earth Observation Satellites: Monitor natural resources, forests, and polar ice. Example: IRS series.
- Military Satellites: Gather security information.
Common Question: Why do we need different types of satellites?
Each type serves specific purposes, from communication to resource management, making satellites essential for modern life.
Orbits of Satellites
Low Earth Orbit (LEO):
- Height: 180–2000 km.
- Used for scientific experiments and atmospheric studies.
- Examples: Hubble Telescope, International Space Station (ISS).
Medium Earth Orbit (MEO):
- Height: 2000–35780 km.
- Examples: Global positioning satellites.
High Earth Orbit (HEO):
- Height: >35780 km.
- Geostationary satellites revolve synchronously with Earth’s rotation, appearing stationary.
Common Question: Why are geostationary satellites used for communication?
They remain fixed relative to Earth, enabling uninterrupted signals for TV and internet.
Satellite Launch Vehicles
- Definition: Rockets used to carry satellites into specific orbits.
- Principle: Based on Newton’s Third Law (Action-Reaction).
- Stages:
- Multiple stages reduce weight by detaching used fuel tanks.
- Examples:
- PSLV (Polar Satellite Launch Vehicle): Used for polar orbits.
- GSLV (Geosynchronous Satellite Launch Vehicle): Used for geostationary orbits.
Common Question: Why are launch vehicles costly?
They involve advanced technology and are often single-use, although reusable vehicles like space shuttles reduce costs.
Space Missions Away from Earth
- Missions aim to study celestial bodies or gather data from beyond Earth’s atmosphere.
- Key Achievements:
- Moon Missions: Luna (USSR), Apollo (USA), Chandrayaan (India).
- Mars Missions: Mangalyaan (India), Mars Rover (USA).
Common Question: What is India’s contribution to Mars exploration?
India’s Mangalyaan mission (2013) was cost-effective and provided valuable data about Mars’ surface and atmosphere.
Notable Astronauts and Contributions
- Yuri Gagarin: First human in space (1961, USSR).
- Neil Armstrong: First human on the Moon (1969, USA).
- Rakesh Sharma: First Indian in space (1984, USSR).
- Kalpana Chawla and Sunita Williams: Indian-origin astronauts contributing to NASA missions.
Common Question: Why are astronauts important for space missions?
Astronauts conduct experiments in space, advancing knowledge and technology.
Space Debris and Management
- Definition: Non-functional satellites, rocket parts, and collision fragments orbiting Earth.
- Problems:
- Risk of collisions with operational satellites.
- Increased difficulty in launching new missions.
- Solutions:
- Designing satellites to deorbit after use.
- Using nets or magnets to collect debris.
Common Question: How does space debris affect future missions?
Debris can damage satellites or spacecraft, posing challenges for space operations.
Space Research Organizations
ISRO (India):
- Notable achievements: Aryabhata, Chandrayaan, Mangalyaan.
- Launch sites: Sriharikota, Thumba.
NASA (USA):
- Notable missions: Apollo, Mars Rovers.
Other Organizations:
- ESA (Europe), Roscosmos (Russia), CNSA (China).
Common Question: Why is ISRO considered a leader in cost-effective space exploration?
ISRO achieves significant milestones with minimal budgets, like the Mangalyaan mission.
Future of Space Exploration
- Interplanetary Travel: Missions to Venus, asteroids, and comets.
- Space Tourism: Private companies like SpaceX making space travel accessible.
- Colonization: Plans for human habitats on Mars and the Moon.
Common Question: How close are we to living on another planet?
While still in development, advancements in technology make colonization a possibility for future generations.
Conclusion:
The chapter on space missions showcases humanity’s progress in exploring the universe. From artificial satellites improving life on Earth to interplanetary missions unlocking the secrets of the cosmos, space exploration is a testament to human curiosity and innovation. By understanding these advancements, students can appreciate the significance of science and technology in shaping the future.
End Note for Students and Teachers:
These comprehensive notes from Chapter 1 to Chapter 10 have been thoughtfully written to help students understand and revise the key concepts of Class 10 Science and Technology with ease. Each topic has been explained in a detailed, clear, and concise manner, ensuring that even complex ideas are simplified for better understanding. Teachers can use these notes as a teaching aid, and students can rely on them for exam preparation and concept clarity. Share these notes with your friends and classmates to help them succeed, and let’s work together to make learning enjoyable and effective for everyone!